Pulling the heartstrings: building vascular networks in human cardiac tissue
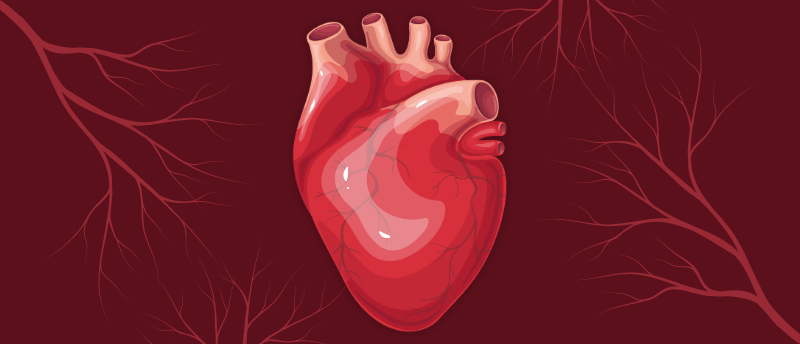
Paul Stankey (left) is a fourth-year PhD student at Harvard School of Engineering and Applied Sciences and the Wyss Institute (both MA, USA), working in Professor Jennifer Lewis’ lab. Jennifer leads a multidisciplinary research group, leveraging expertise in soft matter, cell engineering and additive manufacturing. The lab recently published a paper, of which Paul was first author, detailing how they developed a novel method to 3D print vascular networks embedded in human cardiac tissue.
In this interview, we learn more about this method, the challenges associated with building artificial vasculature and what this work means for growing functional human organs outside the body.
Why are researchers interested in growing organs in the lab?
The main reason why any of us get into this field is because there is an organ shortage, and we have hundreds of thousands of people sitting on a waiting list worldwide, many of whom will sadly die before they can get an organ transplant. Then you must consider that people lucky enough to receive an organ transplant are on immune suppressors for the rest of their lives, increasing their risk of infection. Growing organs in the lab allows us to use cells from the individual receiving the transplant. These organs won’t trigger a false immune response, meaning individuals won’t have to take immune suppressors, and they can live longer, healthier lives.
What are the main challenges associated with the development of lab-grown organs?
There are many. First, lab-grown organs are living things – they’re dynamic – and they require you to build a large and complex structure in a short time.
Second, we’re fabricating across numerous length scales; we work at the cellular level on the order of single microns all the way up to the centimeter scale – when thinking about the thickness of a ventricle – and the tens-of-centimeters scale – when thinking about a full organ. We need to figure out ways to integrate different techniques so we can fabricate micro-vessels, macro-vessels and additional structures to produce a functional organ.
A third challenge is that we have incomplete knowledge of the materials that we’re working with. We don’t know what drives all the biological processes around angiogenic sprouting, cardiac maturation or kidney development, for example.
In summary, we’re engineering living, breathing systems, and we don’t know everything about them yet, which forces us to be creative in how we fabricate different structures.
Why is it so difficult to achieve vascularization?
The length scales make creating vascular networks challenging because we have arteries on the orders of millimeter and centimeter, and these have to connect to capillaries on the scale of tens of microns. One strategy isn’t going to be able to fabricate all those length scales in one go.
A second reason why vascularization is difficult is that we’re working with a brand-new material set. There’s been this fundamental paradigm shift in bioengineering and biofabrication to using living materials as engineering materials. We’re still learning how to work with these biomaterials.
Then, finally, a third reason is that multi-material structures are extremely hard to work with. There aren’t that many manufacturing techniques that can easily integrate multiple materials into pre-defined locations.
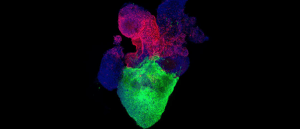
Credit: Aleksandra Kostina
Heart organoid model illuminates key aspect of congenital heart defect pathology
An organoid model of the human heart provides insights into the pathology of diabetes-induced heart defects in newborns. Image shows an organoid with a ventricle-like structure at the bottom, and an proepicardial-like structure at the top.
Can you tell us about the technological developments that are improving our ability to vascularize organs?
Back in 2014, our group published a paper, Kolesky et al., detailing how they used multi-material 3D printing to create channels alongside cells. The channels were created by injecting a material that could then be washed away. This work was on the macrovascular scale – on the order of 500 microns and above.
2019 was really the start of highly cell-dense models, which are what people think about when we say we’re making an organ tissue. This is when the original ‘sacrificial writing in functional tissue’ (SWIFT) technique was developed, which was also published by our lab. The idea here is that you pack lots of cell spheroids together, which form a solid at rest, and then when you draw a needle through these cells – in other words, printing filaments within this cell bath – the cell bath becomes liquid. As you draw the needle through the bath, it heals and locks those vessels into place. It results in a very cell-dense tissue that can be kept alive by these single layer channels that have been drawn into it. But again, these ‘blood vessels’ are channels, rather than structurally defined vessels, which have a wall and lumen.
My work, then, was concerned with developing the actual structure of the blood vessel – the core–shell architecture. In a blood vessel, there’s a shell, which is the wall of the vessel, and then a hollow interior channel, the core. These can be fabricated quite easily, as they are basically straws that run through your tissue as single channels. However, this isn’t super useful for developing organs as vasculature is far more complex. What we figured out with our newest paper was how to connect all those straws together, taking us much closer to creating realistic vasculature and therefore functional organs.
What technologies have you developed to allow you to build this more realistic vasculature in cardiac tissue?
We are bringing together two technologies to do this work. The first technology is called coaxial bio-printing or core–shell bio-printing, which allows us to fabricate the shape of the blood vessel using a nozzle that can produce a single filament with two layers. Using a gelatin ink, we print the core or lumen of the blood vessel; this works because when we print it, it’s cold and solid, but once it’s heated, it liquefies. Wrapped around this gelatin ink is the shell, which is made from collagen and muscle cells. This layer is also printed cold and behaves like a solid. However, it is shear thinning, so you can extrude it out of the nozzle. However, when you heat up this layer, it solidifies, locking those cells in place.
We were able to create branching vascular networks because of the nozzle we developed, which was actually a very simple concept. We took the interior needle where that core ink flows through, and we extended it past the exterior needle, which is where the shell flows through. This allowed us to poke through the shell to connect the core of one vessel to another before connecting the shells.
The second technology that’s allowing us to create realistic vasculature is embedded 3D printing, which facilitates the printing of these blood vessels into living human cells. This works similarly to what was done in 2019 by growing hundreds of thousands of cell spheroids and packing them together. Again, when these cells are at rest, they’re a solid. When you drag your core–shell bio-printing nozzle through the tissue, it liquefies, and then heals itself, locking the blood vessel in place. This technique is called co-SWIFT and produces a cell-dense tissue that displays complex vasculature.
How does this technique translate to smaller or larger scales, to create the range of blood vessels needed for heart function, such as capillaries and arteries?
We’re not quite there yet, but it’s next on our list. We want to be able to build big structures that can be supported by macro-vasculature, but to get these tissues functioning properly, we need to understand biological self-assembly. In other words, we need to figure out how to get cells to build really small-scale architectures for us and then have those connect into the macro-vasculature that we’re 3D printing with co-SWIFT.
What we’ve done so far is take a CT scan from a patient, isolating the left coronary artery and the main branches off that. Then we turned that into a 3D printing path, and we 3D printed it. You can watch a demonstration here; [with reference to the demonstration, featured below] we’re looking up into these 3D structures. You can see that on the left, we’ve printed into living cardiac organoids and on the right, we are printing into a transparent alginate matrix, which behaves exactly the same way as the organoids but makes the vascular networks easier to see. There are more demos on our site.
We have now published co-SWIFT, so anyone can use it. What’s particularly nice about this technique is you don’t need a fancy printer to do it. You can buy a coaxial 3D-printing nozzle, or you can manufacture it yourself. To make our specific nozzle design, you simply have to extend the core needle a little bit past the shell needle to be able to poke through to make these connections. The main consideration to get right is your material properties, and we’ve defined these in the paper.
Do you have any tips for best practice when using this technique?
As I mentioned, it is very sensitive to the material properties, so quality controlling your materials is essential. However, when you’re working with a living matrix, you’re on a short time scale, and it’s expensive and hard to make. You must come up with other ways to quality control your materials without doing destructive rheological testing. There are all sorts of clever techniques you can use to do this. If you’re using the exact same material set we use in the paper, you can use our quality control techniques, but if you’re switching the material set, then I would highly recommend finding a way to quality control your materials.
If there was one thing you could ask for that would help improve our ability to vascularize engineered tissues, what would it be?
As we look towards actually implanting these engineered tissues into animals, and eventually people, we want more robust vessels. With the co-SWIFT technique, we can make vascular shapes and structures. However, the next step is to make these vessels strong enough to be able to withstand blood pressure and make them safe to implant into people.
What can we expect of tissue engineering and organ manufacturing in the future?
As we look at the field in general, I don’t believe it’s a question of if anymore. It’s a question of when we’re going to see this in the clinic. This field is going to start moving faster and faster, but it’s going to be incremental. We’re going to see building blocks coming into the clinic. Then before we know it, we’re going to be working with organ-scale tissues.
Of course, it’s important to manage expectations around what tissue engineering can do, and when we’re actually going to see it. It’s one thing to estimate when we’re going to be able to build organ-scale tissues in the lab, but it’s an entirely different thing to estimate when we’ll see these in the clinic because that requires a great deal of regulation. Within a decade I believe we’ll create a lab-grown organ, and I think that’ll generate a lot of buzz. However, it’s going to be probably another 10 to 20 years before we figure out all the regulation requirements. So, we’ll certainly see this technology utilized within our lifetime, but probably not as soon as we’d like.
The opinions expressed in this interview are those of the interviewees and do not necessarily reflect the views of BioTechniques or Taylor & Francis Group.