Bacteriophages 2.0 — an old solution to a modern problem
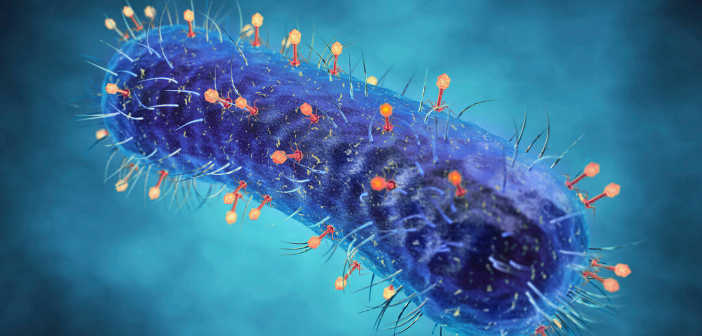
Antibiotics have been our primary defense against bacteria since the mid-20th century. However, they are rapidly becoming less effective as their inappropriate use has selected for resistant bacteria [1]. The rise of antimicrobial resistance has driven the medical and scientific communities to look for alternatives to treat infections caused by these difficult strains. An old solution, discovered over a decade before penicillin, is experiencing a resurgence in interest. Bacteriophages (phages) – viruses that specifically kill bacteria – were discovered in the early 1900s [2]. They are the most abundant entity on the planet, outnumbering their bacterial and archaeal hosts by 10-fold. As obligate intracellular parasites, they must infect a host in order to replicate. They can undergo two life cycles, lysogenic and lytic [3]. Lysogenic phages embed their genetic material in the chromosome of their bacterial hosts where they replicate as part of the genome until host distress (e.g., exposure to DNA damaging agents) triggers their excision and entry into the lytic phase. Lytic phages infect a cell, make multiple copies of themselves, then lyse the host to escape.
The ability of phages to rapidly kill bacteria led soon after their discovery to exploration of their potential as antimicrobial agents for human infections. However, the subsequent identification of broad-spectrum antibiotics led to the abandonment of phages as therapeutics with the exception of a few centers [2]. The exquisite specificity of phages for their bacterial hosts – some can infect only certain strains of a species – means that it is necessary to identify the exact cause of a bacterial infection, then match the infecting organism with appropriate phages. Until recently, it was too challenging to do this in a timely manner. In contrast, antibiotics could be administered ad hoc for infections of unknown etiology. These important drugs changed the face of medicine, allowing us to perform procedures that would have been unthinkable 100 years ago including organ transplants, joint replacement and chemotherapy for cancer.
“Recent advances in diagnostics mean that the cause of an infection can be more rapidly identified than in the past, allowing the timely selection of relevant phages for treatment.”
The bad news is that bacteria evolve quickly, and the indiscriminate use of antibiotics has led to the emergence of multidrug-resistant and even pan-resistant strains [4]. When this unfortunate situation is combined with the lack of new molecules in the discovery pipeline due to withdrawal of most big pharmaceutical companies from antibiotic development [5], we risk reverting to an era where infections may once again be untreatable. Thus, scientists and clinicians are turning back to phages to treat difficult antibiotic-resistant infections. Phages are readily isolated from environmental samples and can be modified to enhance desirable or delete undesirable properties [6]. Recent advances in diagnostics mean that the cause of an infection can be more rapidly identified than in the past [7], allowing the timely selection of relevant phages for treatment. Further, a new appreciation of the importance of the human and animal microbiome to health has prompted a search for more targeted treatments that are less likely to cause collateral damage to benign microbes. Due to their strict host specificity, phages are recognized by regulatory agencies as generally safe for human consumption and are already used in food processing and agriculture to control pathogens [8]. The regulatory landscape for phage treatment of human infections is still evolving as the science matures.
Phages use bacterial surface components as primary receptors to target appropriate bacterial hosts, and mutations leading to loss of those structures can confer phage resistance [8]. Therefore, multiple phages that recognize distinct primary receptors are typically administered as cocktails to minimize the emergence of resistance. However, the evolutionary pressure for loss of specific receptors could be exploited to our advantage if those receptors are important for fitness [2]. For example, many phages use common virulence factors, including lipopolysaccharides, capsules, flagella, pili or teichoic acids as receptors, and alteration (e.g., phase variation) or loss of these factors can lead to reductions in bacterial pathogenicity. Careful design of phage cocktails can thus encourage selection for phage-resistant but avirulent mutants. Phages can also be administered with antibiotics, in some cases resulting in synergy. For example, one study reported that administration of a phage that uses the outer-membrane component of a drug efflux pump as a receptor in combination with antibiotics forced the bacteria to evade the phage through loss of efflux pump expression, with a collateral increase in susceptibility to the antibiotic [10]. A better understanding of how antibiotics impact bacterial physiology during infection is needed to prevent unintentional inhibition of phage replication.
“Although the few phage therapy clinical trials that have been completed yielded underwhelming results – mostly for technical reasons such as accidental underdosing – recent case studies where phages and host bacteria were well matched have demonstrated the incredible potential of phage therapy to treat challenging infections.”
Although the few phage therapy clinical trials that have been completed yielded underwhelming results – mostly for technical reasons such as accidental underdosing [11] – recent case studies where phages and host bacteria were well matched have demonstrated the incredible potential of phage therapy to treat challenging infections [12–14]. Broader implementation of phage therapy will require that certain limitations are addressed. One issue is lack of diversity in phage libraries despite the vast spectrum of phages we suspect are present in natural environments [15]. Enrichment of phages from environmental samples has historically been done using nutrient rich growth conditions [16], favoring the amplification of phages that recognize abundant surface components. However, in the environment and during infection bacteria frequently encounter conditions where specific nutrients, such as iron, are limiting. Adaptations to those limitations include the increased expression levels of cell-surface receptors for uptake of iron-binding siderophores [17], and phages that use such receptors as their primary targets have been identified. Enriching for phages using bacteria that are grown in more host-like conditions will increase diversity, which in turn is likely to cover more strains. Similarly, enriching for phages using bacterial deletion mutants that lack the most common primary receptors will help to amplify rare phages that recognize alternate receptors that may otherwise be masked. Other variables such as pH and competition from other bacteria could affect the types of surface receptors expressed under clinically relevant conditions. Conversely, production of genetically identical phages from the same bacterial host grown under various conditions (such as in liquid versus attached to surfaces as a biofilm, which results in changes in gene expression [18]) may result in important differences that have not been carefully explored. Phages can carry genes encoding toxins or resistance elements, so detailed characterization of prospective therapeutic phages by DNA sequencing is important to ensure that they are free of potentially harmful elements [19].
Another drawback to the wider implementation of phage therapy is our rudimentary understanding of phage–bacteria dynamics in the context of infection and the human immune response. Unlike antibiotics, phages replicate and evolve in parallel with their bacterial hosts [20]. The ability to replicate can be an advantage as it can minimize dosing requirements due to self-amplification. The potential for lysogeny must also be considered; in some studies, therapeutic phages have been engineered to remove genes encoding repressor proteins that maintain the phages in a lysogenic state [13].
An alternate approach to managing the potential for undesirable phage evolution is to use phage components rather than intact particles. Several groups and some companies are developing therapeutics based on lysins, enzymes produced by lytic phages to degrade the cell wall of their host bacteria [21]. One such enzyme, (CF-301) targeting methicillin-resistant Staphylococcus aureus (MRSA) has entered Phase II clinical trials to treat hospitalized patients with MRSA bacteremia or endocarditis. Its developer, Contrafect (NY, USA), has also received funding through the CARB-X program to identify lysins effective against Gram-negative species.
Over the last century we have taken full advantage of the antibiotics produced by soil bacteria to solve important medical problems, increasing our quality and length of life. As we face the potential failure of these critically important molecules, it is gratifying to see renewed interest in bacteriophages, nature’s other tailor-made solution to the problem of antibiotic-resistant bacteria. Beyond the pressing concern of untreatable infections, detailed studies of phages have the potential to uncover exciting new biology, exemplified by the transformative discovery of CRISPR–Cas DNA editing systems and phage-encoded anti-CRISPR defenses [22]. With new molecular tools in hand, we are entering a fascinating new era of phage research.
Acknowledgements
Phage research in the Burrows lab is funded by grants from the Canadian Institutes of Health Research and the David Braley Centre for Antibiotic Discovery at McMaster University.
- Blair JM, Webber MA, Baylay AJ, Ogbolu DO, Piddock LJ. Molecular mechanisms of antibiotic resistance. Nat. Rev. Microbiol. 13, 42–51, doi:10.1038/nrmicro3380 (2015).
- Nobrega FL, Costa AR, Kluskens LD, Azeredo J. Revisiting phage therapy: new applications for old resources. Trends Microbiol. 23, 185–191, doi:10.1016/j.tim.2015.01.006 (2015).
- Monteiro R, Pires DP, Costa AR, Azeredo J. Phage therapy: going temperate? Trends Microbiol. 27, 368–378, doi:10.1016/j.tim.2018.10.008 (2019).
- Wright GD. Antibiotic adjuvants: rescuing antibiotics from resistance. Trends Microbiol. 24, 862–871, doi:10.1016/j.tim.2016.06.009 (2016).
- Rex JH, Outterson K. Antibiotic reimbursement in a model delinked from sales: a benchmark-based worldwide approach. Lancet Infect. Dis. 16, 500–505, doi:10.1016/S1473-3099(15)00500-9 (2016).
- Chen Y, Batra H, Dong J, Chen C, Rao VB & Tao P. Genetic engineering of bacteriophages against infectious diseases. Front. Microbiol. 10, 954, doi:10.3389/fmicb.2019.00954 (2019).
- Tsalik EL, Bonomo RA, Fowler VG Jr. New molecular diagnostic approaches to bacterial infections and antibacterial resistance. Ann. Rev. Med. 69, 379–394, doi:10.1146/annurev-med-052716-030320 (2018).
- Moye ZD, Woolston J, Sulakvelidze A. Bacteriophage applications for food production and processing. Viruses. 10, doi:10.3390/v10040205 (2018).
- Bertozzi Silva J, Storms Z, Sauvageau D. Host receptors for bacteriophage adsorption. FEMS Microbiol. Lett. 363, doi:10.1093/femsle/fnw002 (2016).
- Chan BK, Sistrom M, Wertz JE, Kortright KE, Narayan D,Turner PE. Phage selection restores antibiotic sensitivity in MDR Pseudomonas aeruginosa. Sci. Rep. 6, 26717, doi:10.1038/srep26717 (2016).
- Jault P, Leclerc T, Jennes S et al. Efficacy and tolerability of a cocktail of bacteriophages to treat burn wounds infected by Pseudomonas aeruginosa (PhagoBurn): a randomised, controlled, double-blind Phase 1/2 trial. Lancet Infect. Dis. 19, 35–45, doi:10.1016/S1473-3099(18)30482-1 (2019).
- Schooley RT, Biswas B, Gill JJ, Hernandez-Morales A et al. Development and use of personalized bacteriophage-based therapeutic cocktails to treat a patient with a disseminated resistant Acinetobacter baumannii infection. Antimicrob. Agents. Chemother 61, doi:10.1128/AAC.00954-17 (2017).
- Dedrick RM, Guerrero-Bustamante CA, Garlena RA et al. Engineered bacteriophages for treatment of a patient with a disseminated drug-resistant Mycobacterium abscessus. Nat. Med. 25, 730–733, doi:10.1038/s41591-019-0437-z (2019).
- Kuipers S, Ruth MM, Mientjes M, de Sevaux RGL, van Ingen J. Successful treatment of chronic relapsing urinary tract infection with bacteriophages in a renal transplant recipient – a Dutch case report. Antimicrob. Agents Chemother. doi:10.1128/AAC.01281-19 (2019).
- Hatfull GF. Dark matter of the biosphere: the amazing world of bacteriophage diversity. J. Virol. 89, 8107–8110, doi:10.1128/JVI.01340-15 (2015).
- Mattila S, Ruotsalainen P & Jalasvuori M. On-demand isolation of bacteriophages against drug-resistant bacteria for personalized phage therapy. Front. Microbiol. 6, 1271, doi:10.3389/fmicb.2015.01271 (2015).
- Wilson BR, Bogdan AR, Miyazawa M, Hashimoto K, Tsuji Y. Siderophores in iron metabolism: from mechanism to therapy potential. Trends Mol. Med. 22, 1077–1090, doi:10.1016/j.molmed.2016.10.005 (2016).
- Hall CW, Mah TF. Molecular mechanisms of biofilm-based antibiotic resistance and tolerance in pathogenic bacteria. FEMS Microbiol. Rev. 41, 276–301, doi:10.1093/femsre/fux010 (2017).
- Philipson CW, Voegtly LJ, Lueder MR et al. Characterizing phage genomes for therapeutic applications. Viruses 10, doi:10.3390/v10040188 (2018).
- Chaturongakul S, Ounjai P. Phage-host interplay: examples from tailed phages and Gram-negative bacterial pathogens. Front. Microbiol. 5, 442, doi:10.3389/fmicb.2014.00442 (2014).
- Raz A, Serrano A, Hernandez A, Euler CW & Fischetti VA. Isolation of phage lysins that effectively kill Pseudomonas aeruginosa in mouse models of lung and skin infection. Antimicrob. Agents Chemother. 63, doi:10.1128/AAC.00024-19 (2019).
- Nakamura M, Srinivasan P, Chavez M et al. Anti-CRISPR-mediated control of gene editing and synthetic circuits in eukaryotic cells. Nat. Commun. 10, 194, doi:10.1038/s41467-018-08158-x (2019).