See deeper into the brain with multi-photon imaging
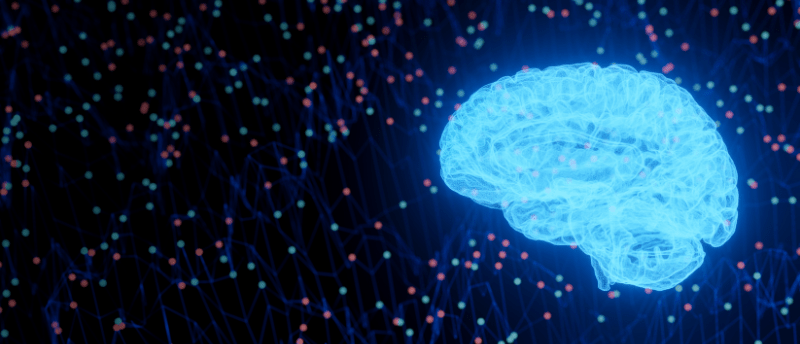
Robert Prevedel is a researcher at the European Molecular Biology Laboratory (EMBL; Heidelberg, Germany), whose lab focuses on developing light microscopy-based techniques for a variety of applications, including neuroimaging techniques that capture brain activity and structure in different model organisms, focusing especially on mouse models.
We caught up with Prevedel at the Federation of European Neuroscience Societies Forum (FENS; 9th–13th July 2022) held in Paris (France) and discussed multi-photon microscopy, photoacoustic imaging, the challenges of deep brain imaging and the future of neuroimaging.
What did you present at FENS this year?
We organized the technical workshop on multi-scale optical microscopy for brain imaging in mice and other model organisms. I also gave a talk where I presented some recent results from my lab, on a microscope we developed for deep brain imaging using three-photon microscopy and adaptive optics. This technique is used to obtain high-resolution images deep inside tissues.
Can you explain some of the techniques that you use for deep brain imaging?
We use multi-photon microscopy as a method to excite fluorophores that are usually expressed inside brain cells in mice. We use three-photon microscopy – i.e. you need three photons to combine to raise the energy of the fluorophore – because of its higher non-linearity, which helps remove out-of-focus background signals and improve the image quality and contrast. Additionally, this technique uses near-infrared laser light. This longer wavelength of light is usually less attenuated and less scattered inside tissues, which means that you can take images much deeper inside the brain.
Another aspect of the technique is adaptive optics. This is a concept that’s actually borrowed from astronomy and allows us to correct aberrations caused by light interacting with living tissues. With adaptive optics, we can sort of reverse that process and cancel out these optical aberrations, retaining a really sharp image of the brain. With this sharper focus, you are not only getting higher signal levels – which is always good – but, you also get sharper images, meaning a higher resolution. This then allows us to study brain structures in finer detail, so we can see individual dendrites and synapses that would be lost with more standard approaches.
What are the challenges of deep brain imaging in biological tissues?
A big challenge is that when light interacts with brain tissues, the light is attenuated, it’s scattered and it’s absorbed, all of which contributes to a blurry image. If you want to image deep in the brain, the excitation light from the laser is lost and that also results in a blurry image. Another challenge when imaging a living mouse brain is that heart pulsation causes tiny vessels in the brain to rhythmically dilate, which leads to tissue motion. This motion shakes the brain a little bit and that leads to, again, blurry and distorted images.
In our recent work, we used active methods such as electrocardiography gating (ECG). We recorded the electrocardiogram of the mouse and then synchronized the microscopy to it so that it would take images when the brain would not move as much, giving us cleaner images without motion artifacts or blurry areas.
The development of pain perception in early life
In this interview, we chat to Rebeccah Slater, a professor of Pediatric Neuroimaging in the Department of Pediatrics (Oxford University, UK), about her research on neonatal pain perception and her involvement in FENS 2022.
How does multi-photon microscopy work to improve the contrast and signal intensity?
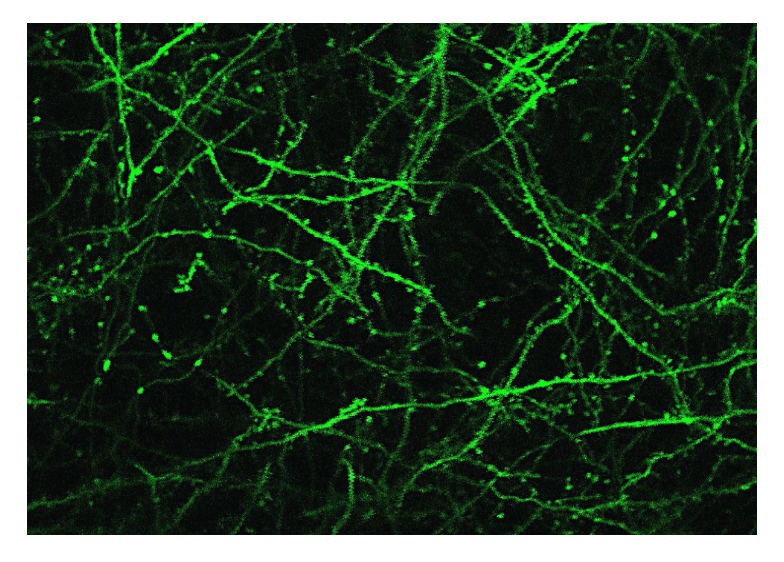
In vivo image of neurons using three-photon microscopy. Image used with permission from Prevedel’s lab.
The advantage of our methodology is that with multi-photon excitation we can really confine the area from where we excite the fluorophore, so the background of the image is less out-of-focus, and the image has less of a ‘halo’ around it. This means the image is cleaner and has a higher contrast, so the fluorescing neuron is much brighter compared to the background. Similarly, with adaptive optics, we can really focus the light on the smallest possible area, which means that we can see dendrites and synapses much clearer.
Can you explain the technique you’re developing using photoacoustic imaging and which computational approaches you’re incorporating?
Photoacoustics is a hybrid of two modalities – light microscopy and ultrasound – in which you excite acoustic waves using light. Light is highly scattered inside brain tissues, but ultrasound is not, which is why we can see deep into our own body with ultrasound; however, ultrasound has a poorer resolution compared to light microscopy. For this to work, we shine light into the tissue, and it is absorbed by molecules, which expand and give off tiny pressure waves. In the lab, we mostly work on novel optical techniques to detect those pressure waves sensitively, which in turn allows us to reconstruct and detect molecules that are very deep inside the tissue – in principle up to 10mm. This is much deeper than in the multi-photon work that I presented today, where the limit is around 1–2mm.
We don’t develop new computational algorithms, but we use tomographic principles to relocate the sound waves and then reconstruct an image. In microscopy, you excite a given point and then collect all the fluorescence and put it in a pixel. In photoacoustic tomography, you basically detect the sound waves from multiple different places, at different angles, and that allows you to triangulate where the signal came from. Without these computational methods, it would be impossible to tell where the sound waves originated from, and it would be impossible to obtain an actual image.
Is reproducibility an area of concern in your work and how is your lab working to overcome the reproducibility crisis?
It’s not a big issue in our work. For us in technology development, the fact that this is highly specialized work makes it very difficult to reproduce experiments just by describing the method in the paper. Even if you give all the information in the supplementary material that should, in principle, allow someone to reproduce it, it’s still technically very challenging. You need lots of training or exact expertise, and that is often missing.
In our publications, we regularly give out the source code of the computational algorithms and make detailed part lists of hardware that we used to build up the microscope and supply computer-aided design (CAD) drawings that can be used to manufacture custom parts. In the end, of course, somebody still needs to take all that material and put it together. There’s only that much that you can do offline and not in person, and we are well aware of that. There are separate programs in our field aiming to address this and we try to participate in these as well.
I hope that there’s no real reproducibility crisis in microscopy itself, but it’s not easy to disseminate and get certain technologies to every lab, for example, not every lab can just build their own three-photon microscope.
What do you think will be the biggest breakthrough in imaging in the next 5 years?
Looking back in time, I think there’s been tremendous progress in the area of neural activity recording. Today, at the FENS forum, we saw talks where people showed that we are now able to record thousands to hundreds of thousands to even 1 million neurons in an awake mouse. I think this is a fantastic achievement because 5 years ago, that number was around 1000 and now, it’s 1 million. If the field keeps on growing like this, at one point it will certainly be possible to image almost the entirety of a mouse brain.
I think one of the biggest challenges currently is a lot of neuroimaging methods indirectly look at the activity of neurons. In optical microscopy, we’re actually detecting calcium and not the underlying electrical signal of the brain cells. So, the next challenge for our field is achieving similar recording capabilities and similar microscopes that work not only for calcium probes but also for voltage probes, which directly sense the membrane potential of the neuron. Hopefully, labs will keep pushing the boundary and be able to get recordings of similar quality with a similarly huge number of neurons – not just for calcium, but for voltage.